Chapter 13 – Organic Chemistry
Introduction
Organic chemistry is the study of carbon based compounds. The structural and genetic materials of living organisms are organic compounds. Many of the substances that we encounter on a daily basis are organic compounds: drugs, plastics, textiles, dyes, paper, food, vitamins, etc. Because of the manner in which carbon atoms can bond to one another, the number of organic compounds is enormous. In this chapter, we explore some of the fundamental topics of the structure and reactivity of several classes of organic compounds by applying our knowledge of Lewis structures, resonance, and Lewis acid-base theory.13.1 Hydrocarbons
Introduction
We begin our study of organic chemistry with two classes of compounds that contain only carbon and hydrogen.Prerequisites
-
•5.8 Formal Charge and Oxidation State (Draw Lewis structures.)
Objectives
-
•Name and draw the two simplest alkanes.
-
•Draw line-wedge-dash, Lewis, condensed, and skeletal structures of alkanes.
-
•Convert skeletal structures into molecular formulas.
-
•Distinguish between a straight or continuous chain and a branched chain.
-
•Write the equation for the combustion of an alkane.
-
•Define an alkene and draw and name the two simplest alkenes.
-
•Draw the isomers of an alkene.
-
•Define a polyene and explain why many polyenes absorb visible light.
-
•Define alkynes and discuss their isomers.
-
•Distinguish between saturated and unsaturated hydrocarbons.
Alkanes
13.1-1. Definition
Carbon atoms always have four bonds: four single bonds, two single and one double bond, two double bonds, or a single bond and a triple bond. Although carbon is the basic building block, organic compounds usually have hydrogen atoms as well. Oxygen and nitrogen are also common, but almost any other element can be found in organic compounds. In this section, we deal with the class of compounds known as hydrocarbons, which are compounds that contain only carbon and hydrogen. Alkanes are the simplest organic compounds. All of the carbon atoms in an alkane are sp3 hybridized and tetrahedral, and all bonds are sigma bonds, which makes alkanes relatively unreactive. They have the general formula CnH2n + 2, where n is an integer. Table 13.1 shows the three simplest alkanes.Alkane | Structure |
---|---|
Methane, CH4 | ![]() |
Ethane, C2H6 | ![]() |
Propane, C3H8 | ![]() |
Table 13.1: The Simplest Alkanes
13.1-2. Structures
Carbon atoms always have four bonds in their compounds.
-
•Carbon atoms form their backbone.
-
•Carbon atoms always have four bonds to them.
-
•There are usually many C–H bonds present.

Figure 13.1a: Representations of Propane: Wedge-Dash

Figure 13.1b: Representations of Propane: Lewis Structure

Figure 13.1c: Representations of Propane: Condensed Structure

Figure 13.1d: Representations of Propane: Skeletal Structure
13.1-3. Molecular Formulas for Skeletal Structures
Neither carbon nor hydrogen atoms are shown explicitly in a skeletal structure.
-
•Carbon atoms are represented as intersections or ends of lines.
-
•The number of hydrogen atoms attached to a carbon atom is equal to four minus the number of bonds involving the carbon atom because carbon atoms are always involved in four bonds. Note that double and triple bonds count as 2 and 3 bonds, respectively.
Exercise 13.1:
Write the molecular formula for the given skeletal structures. Note that the order of elements in the formula should be C then H then O. (Indicate any subscripted characters with an underscore (_) and any superscripted characters with a carat (^). For example, NH_4^1+ for NH41+. Omit any spaces.)
13.1-4. Chains
Isomers are different molecules with the same formula.

Figure 13.2a: Isomers of Butane: Straight Chain
Lewis (a), condensed (b), and skeletal (c) representations of the straight or continuous chain isomer: It is a straight chain isomer because none of the carbon atoms are connected to more than two other carbon atoms.

Figure 13.2b: Isomers of Butane: Branched Chain
It is a branched isomer because there are three carbon atoms connected to the central carbon atom, so the chain branches at that position.
13.1-5. Combustion
Alkanes are found primarily in natural gas (mainly CH4 and C2H6) and petroleum. They are relatively unreactive, but they do burn well. Burning is a rapid combustion, i.e., the reaction with oxygen. The products of the combustion of an alkane are CO2 and H2O. Table 13.2 shows the heats of combustion for four common alkanes.Alkane | Use | Combustion Reaction | Heat of Combustion (kJ/mol) |
---|---|---|---|
methane | natural gas furnace | CH4 + 2 O2 → CO2 + 2 H2O | –891 |
propane | propane tank | C3H8 + 5 O2 → 3 CO2 + 4 H2O | –2220 |
butane | cigarette lighters | C4H10 + 6.5 O2 → 4 CO2 + 5 H2O | –2855 |
octane | automobile fuel | C8H18 + 12.5 O2 → 8 CO2 + 9 H2O | –5494 |
Table 13.2: Combustion of Some Common Alkanes
13.1-6. Octane Ratings
Each atom in a C–H or C–C bond retains one of the bonding electrons during combustion. The result is two species, called free radicals, that have unpaired electrons. Free radicals are highly reactive, but they are stabilized when the unpaired electron is on an atom that is attached to other carbon-containing groups. Thus, free radicals formed from highly branched carbon atoms are more stable and less reactive than those formed from straight chain hydrocarbons.
Figure 13.3: Free Radicals
Bond cleavage of a C–C bond during combusion produces two free radicals. The radicals are stabilized when the carbon atom with the unpaired electron is bound to one or more carbon atoms, so (CH3)3C· is more stable and less reactive than H3C·.

Figure 13.4: Reference Molecules Used to Determine Octane Ratings
Alkenes
13.1-7. Definition
Organic compounds that contain carbon-carbon double bonds are called alkenes. The carbon atoms involved in the double bond are sp2 hybridized. The two simplest alkenes are ethene (C2H4) and propene (C3H6). Alkenes in which the position of the double bond is different are different molecules. However, care must be taken to be certain that the two positions are indeed different and not just a different view of the same molecule.
Figure 13.5a: a) Lewis, b) Condensed, and c) Line-Wedge Representations of Ethene
Note that the p orbitals and the pi interaction are shown in the line-wedge structure.

Figure 13.5b: a) Lewis, b) Condensed, and c) Skeletal Structures of Propene
13.1-8. Butene
The double bond in C4H8 can be placed in three different positions (after the first or second carbon in a straight chain or after the first carbon of a branched chain) to produce three isomers. In addition, groups cannot rotate about double bonds like they can about single bonds because the rotation would break the pi bond. Consequently, the butene with two carbon atoms (two CH3 groups) on the same side of the double bond is different than the one with the two carbon atoms on opposite sides. We will discuss this type of isomer in more detail later. The four isomers of C4H8 are derived below by starting with propene as follows.
Figure 13.6: Possible Positions of the Fourth Carbon Atom in Butene
The skeleton of C3H6 is highlighted in blue. The fourth carbon (CH3 group) of C4H8 can be placed in any of the labeled positions to produce a total of four different molecules (isomers).
Position of 4th C | Skeletal Structure | Comments |
---|---|---|
1 | ![]() |
This is a straight chain alkene with double bond after the second carbon atom. In this isomer, the two CH3 groups are on the same side of the double bond. |
2 | ![]() |
This is also a straight chain alkene with double bond after the second carbon atom. In this isomer, the two CH3 groups are on opposite sides of the double bond. |
3 | ![]() |
This isomer is the only branched isomer. |
4 | ![]() |
This is the only straight chain isomer in which the double bond involves one of the end carbons. |
Table 13.3: The Isomers of Butene
13.1-9. Polyenes
Organic dyes are polyenes with alternating single and double bonds.





Figure 13.7a: Skeletal Structures of Two Polyenes: Beta Carotene
Beta carotene gives carrots their orange color and is oxidized to vitamin A in the body. Its color is due to the large number of alternating single and double bonds (21).

Figure 13.7b: Skeletal Structures of Two Polyenes: Congo Red
Congo red was one of the first dyes for cotton. It has 41 bonds involved in the pi system. The polar NH2 and SO31– groups form hydrogen bonds to the cellulose in the cotton fiber, which keeps the dye from washing out.
Alkynes
13.1-10. Definition
Alkynes are hydrocarbons that contain at least one triple bond. The simplest alkyne is C2H2, which is ethyne, or more commonly acetylene.
Figure 13.8
13.1-11. Isomers
As with alkenes, different positions of the multiple bond can lead to different isomers. However, the positions must be different viewed from either end of the molecule. For example, there are three ways to draw butyne, but there are only two isomers. The two molecules on the left of Figure 13.9 are the same molecule as the triple bond in each is located on a terminal carbon atom. The molecule on the right is the isomer of the molecule on the left.
Figure 13.9: Isomers of Butyne
13.1-12. Hydrogenation and Saturation
Carbon atoms with four sigma bonds are saturated carbons, while those with less than four sigma bonds are unsaturated carbons.
unsaturated hydrocarbon | + | hydrogen | → | saturated hydrocarbon |
---|---|---|---|---|
![]() |
+ |
3 H2 |
→ |
![]() |
Multiple bonds (double and triple) are unsaturated. The more multiple bonds a compound has, the higher is its degree of unsaturation. Unsaturated compounds can be saturated by hydrogenation. | One H2 is required for each double bond. | All C–C bonds are single bonds in the hydrocarbon above, so it is saturated. |
13.2 Naming Simple Hydrocarbons
Introduction
Understanding the nomenclature of alkanes, alkenes, and alkynes will help you understand some of the diversity in organic molecules that will be discussed later in the chapter.Prerequisites
-
•5.8 Formal Charge and Oxidation State (Draw Lewis structures.)
-
•6.4: Valence Bond Theory and Hybridization (Determine the hybridization of an atom in a Lewis structure.)
Objectives
-
•Give the common root names and the endings for alkanes and alkenes.
-
•Name straight chain alkanes.
-
•Identify and name the two simplest alkyl groups.
-
•Name branched alkanes with a single alkyl group.
-
•Name branched alkanes with two alkyl groups.
-
•Name straight chain alkenes and alkynes.
13.2-1. Root Names
The name of a hydrocarbon is based on the name of the longest continuous chain it contains.
-
•Alkanes end in ane.
-
•Alkenes end in ene.
-
•Alkynes end in yne.
# C atoms | Root Name | Name of Alkane | Name of Alkene |
---|---|---|---|
1 | meth | methane | |
2 | eth | ethane | ethene |
3 | prop | propane | propene |
4 | but | butane | butene |
5 | pent | pentane | pentene |
6 | hex | hexane | hexene |
7 | hept | heptane | heptene |
8 | oct | octane | octene |
9 | non | nonane | nonene |
10 | dec | decane | decene |
Table 13.4: Root Names for Organic Compounds
13.2-2. Naming Organic Molecules
Exercise 13.2:
To name a straight or continuous chain alkane, indicate the number of carbon atoms it contains with the appropriate root name and add -ane. For example, C10H22 contains ten carbon atoms, so its root name is dec, and it is an alkane, so -ane is added to the root name. Thus, C10H22 is decane.
Name the following straight or continuous chain alkanes.

o_octane_s
All three molecules contain eight carbon atoms in a continuous chain. Therefore, they are all octane. Note that all three structures represent the same molecule and emphasize the dynamic nature of the structures of alkanes.

13.2-3. Alkyl Groups
An alkyl group is formed by removing an H atom from an alkane. They are the side chains (not on the longest continuous chain) of a hydrocarbon.
Formula of Alkyl Group | Name of Alkyl Group |
---|---|
–CH3 | methyl |
–C2H5 | ethyl |
Table 13.5: The Simplest Alkyl Groups
The dash before the formula indicates where they bond to a longer chain. Propyl, butyl, pentyl, etc., groups also exist, but there are more than one of each of these alkyl groups. For example, there are two different propyl groups that differ by the position of the removed hydrogen, which can come from a terminal carbon atom or from the middle carbon atom. We restrict our discussion of naming organic compounds to compounds that contain methyl and/or ethyl groups to avoid this complexity.
13.2-4. Naming Alkanes
Branched alkanes have side chains, so the name of a branched alkane specifies the longest continuous chain and the identity and position of the side chains (alkyl groups). Although there can be many side chains of varying lengths, we restrict our discussion of branched alkanes to those with one or two side chains, which will be either a methyl or an ethyl group. The position of the alkyl group is indicated by the number of the carbon atom to which it is attached. Thus, the carbon atoms in the longest chain must be numbered. To number the longest continuous chain, start at the end that produces the smallest number for the side chain. To name branched alkanes:-
1Identify the longest chain, select the appropriate root name, and add ane to indicate an alkane.
-
2Number the carbon atoms of the longest continuous chain starting at the end that produces the smaller number for the carbon atom to which the alkyl group is attached.
-
3Identify the side chain and its position.
-
4Name the alkane as the number of the position of the side chain, a hyphen, and then the name of the alkyl group followed by the name obtained in step 1.
As an example, we will follow the steps above to name the following compound.

1
Identify the longest continuous chain.

The longest chain contains six carbon atoms, so this is a hexane.
2
Number the longest continuous chain so that the side chain has the lowest number.

Numbering the chain from left to right places the side chain on carbon 4, but numbering it from right to left puts the side chain on carbon 3. Thus, the chain is numbered from right to left to produce the smaller number for the alkyl group.
3
Identify the side chain and its position.

The side chain contains a single carbon atom, so it is a methyl group. It is attached to carbon number 3.
4
Name the compound by combining the name of the longest chain with the position and identity of the side chain.

3-methylhexane
13.2-5. Naming Alkanes Exercise
Practice naming branched alkanes by naming the three organic compounds in Exercise 13.3. The root names can be found in Table 13.4.
Exercise 13.3:
Answer the questions for each of the branched alkanes.
How many carbon atoms are in the longest continuous chain?
5_0__
There are five carbons in the longest chain.

What is the name of the longest continuous chain?
o_pentane_s
A five-carbon chain is named as a pentane.

What is the name of the alkyl group on the longest chain?
o_methyl_s
The alkyl group contains only one carbon atom, so it is a methyl group.

What is the position of the alkyl group (number of carbon)?
3_0__
The methyl group is attached to carbon number three.

What is the name of the compound?
o_3-methylpentane_s
The compound is 3-methylpentane.

How many carbon atoms are in the longest continuous chain?
8_0__
There are eight carbons in the longest chain.

What is the name of the longest continuous chain?
o_octane_s
An eight-carbon chain is named as an octane.

What is the name of the alkyl group on the longest chain?
o_ethyl_s
The alkyl group contains two carbon atoms, so it is an ethyl group.

What is the position of the alkyl group (number of carbon)?
4_0__
The ethyl group is attached to carbon number four. Remember that the chain must be numbered from the end that produces the smallest number for the side chain position.

What is the name of the compound?
o_4-ethyloctane_s
The compound is 4-ethyloctane.

How many carbon atoms are in the longest continuous chain?
9_0__
There are nine carbons in the longest chain.

What is the name of the longest continuous chain?
o_nonane_s
A nine-carbon chain is named as a nonane.

What is the name of the alkyl group on the longest chain?
o_methyl_s
The alkyl group contains one carbon atom, so it is a methyl group.

What is the position of the alkyl group (number of carbon)?
2_0__
The methyl group is attached to the second carbon atom in the chain.

What is the name of the compound?
o_2-methylnonane_s
The compound is 2-methylnonane.

13.2-6. Naming Alkanes with Multiple Alkyl Groups
To name alkanes with more than one side chain, simply place the names of the alkyl groups in alphabetical order separated by hyphens in front of the root name. For example, 3-ethyl-2-methylheptane is a seven carbon continuous chain with a C2H5 group on the third carbon and a CH3 group attached to the second carbon. If two identical groups are attached to the same carbon, the number of the position is repeated with a comma separator, and the number of groups is given by a prefix, as in 2,2-dimethylheptane.
Exercise 13.4:
Name the following compounds.
13.2-7. Naming Alkenes and Alkynes
Alkenes are numbered so that the C=C double bond has the lowest number possible.
-
1Number the chain so as to give the carbon atoms in the double or triple bond the lowest number.
-
2Specify the chain length with the appropriate root name and add -ene to indicate an alkene or -yne to indicate an alkyne.
-
3Indicate the position of the double or triple bond by the number of the carbon atom in the bond with the lower number in the chain.
-
4The name is the position of the bond as determined in step 3 followed by a hyphen and then the root name determined in step 2.
Exercise 13.5:
Name the following straight or continuous chain alkenes.
13.3 Isomers
Introduction
We have seen that there can be several organic compounds with the same formula. Compounds with the same formula are called isomers. Isomers can have very different chemical and physical properties. In this section, we introduce two broad classifications of isomers.Prerequisites
Objectives
-
•Draw the constitutional isomers with a given molecular formula.
-
•Draw all of the constitutional isomers of an alkane.
-
•Identify stereoisomers.
-
•Name the two types of stereoisomers.
-
•Distinguish between cis and trans isomers.
-
•Describe the molecular process responsible for vision.
-
•Identify stereocenters.
-
•Identify enantiomers.
Constitutional
13.3-1. Constitutional Isomers
Constitutional isomers differ in their connectivities.
-
•Ethers contain the C–O–C connectivity.
-
•Alcohols contain the C–O–H connectivity.

Figure 13.10: Isomers of C3H8O
The three isomers of C3H8O: Isomer (a) is an ether, and isomers (b) and (c) are alcohols.
C–O–C linkage, so they are ethers, while the remaining structures all contain a hydroxyl group, which makes each of them alcohols. For C12H26O, there are well over one hundred isomers! The existence of so many constitutional isomers gives rise to a wealth of structural diversity in organic chemistry. While there is no simple mathematical relationship between the molecular formula and the number of constitutional isomers, you can be certain that molecules with a large number of carbon atoms will have a large number of constitutional isomers.

Figure 13.11: Isomers of C4H10O
The six isomers of C4H10O: Isomers (a) and (b) are ethers, but the others are alcohols.
13.3-2. Isomers of Hexane
Follow these steps to determine the constitutional isomers of an alkane.-
1Start by drawing the straight chain isomer.
-
2Remove one carbon atom from the straight chain produced above and place the removed carbon (methyl group) on each carbon of the resulting chain that produces a different isomer.
-
•The methyl group cannot be placed on a terminal carbon atom as that would lead to the same chain as in the previous step.
-
•It does not matter which way the carbon atoms are numbered, so placing a methyl group on each of the carbon atoms that are next to the end carbon produces the same compound.
-
-
3Remove a second carbon atom and place the two methyl groups or one ethyl group on the remaining chain. The ethyl group cannot be placed on a terminal carbon as that would produce the longest chain obtained in step 1, and it cannot be placed on the second carbon as that would produce one of the isomers produced in step 2.
-
4Keep removing one carbon atom until you get to a length where no new isomers can be obtained.
We now follow these steps to draw the constitutional isomers of C6H14.
1
6-carbon chain

The straight chain isomer is hexane.
2
5-carbon chains

The methyl group can be placed on a carbon that is next to the end or in the middle to produce two isomers.
3
4-carbon chains

Two carbons must be added to a four-carbon chain. An ethyl group cannot be used because no matter where it is placed, it lengthens the chain and produces one of the isomers of the 5- or 6-carbon chains. The two methyl groups can be placed in two ways: one group on each of the two interior carbons or both groups on the same interior carbon.
4
3-carbon chains
There are no isomers of C6H14 with longest chains of less than four carbons. Thus, there are five isomers of C6H14.
13.3-3. Isomers of Heptane - an Exercise
Draw the eight isomers of the alkane C7H16 by adding methyl groups to the longest chain.Your browser must have the Flash plug-in installed to do this example.
Stereoisomers
13.3-4. Definitions
Stereoisomers have the same connectivities, but they differ in the spatial arrangement of their atoms. There are two types of stereoisomers.-
•Geometric isomers: Different spatial arrangement of atoms occurs due to the presence of a bond that cannot be twisted completely. We consider only the case of the double bond.
-
•Enantiomers: Different spatial arrangement of atoms occurs due to the presence of a carbon atom that has four different groups attached to it.
13.3-5. Bond Twisting
Groups can rotate relative to one another about a single bond, but not about a double or triple bond.

Figure 13.12: Rotation can occur about single bonds, but not about double bonds.
(a) The two structures of C2H4F2 are not isomers because the groups can rotate around the single bond. (b) The two structures of C2H2F2 are isomers because the groups cannot rotate around a double bond.
13.3-6. Cis and Trans Isomers
Two atoms on the same side of a double bond are said to be cis, while two atoms on opposite sides of the double bond are said to be trans.
Exercise 13.6:
Consider the following three isomers of C2H2F2.
One has no geometric isomer but is a constitutional isomer of the other two. The other two are geometric isomers of one another—one is cis and one is trans. Indicate which is the constitutional isomer and identify the cis and trans geometric isomers.

-
Isomer A:
-
-
-

-
Isomer B:
-
-
-

-
Isomer C:
-
-
-

13.3-7. Retinal
The vision process is initiated by a cis-trans isomerization.

Figure 13.13a: Cis-Trans Isomerization in Retinal: Cis Isomer
The cis isomer of retinal is found in the eye when it is dark.

Figure 13.13b: Cis-Trans Isomerization in Retinal: Trans Isomer
Absorption of a photon promotes a π → π* transition, so the bond order is reduced in the excited state. The weakened bond can then rotate to convert the cis isomer into the trans isomer shown.
13.3-8. Naming Cis and Trans Isomers Exercise
To indicate the isomer of an alkene, place "cis-" or "trans-" in front of the name of the alkene.
Exercise 13.7:
Name the following compounds.
number of carbon atoms in longest chain
5_0__
There are five carbon atoms in the chain.

position of double bond
2_0__
Always number from the side that gives the double bond position the smallest number.

isomer (cis or trans)
o_cis_s
The carbon-carbon bonds adjacent to the double bond are on the same side of the double bond.

name
o_cis-2-pentene_s
A five-carbon alkene with the double bond after the second carbon and the C–C bonds on the same side of the double bond is cis-2-pentene.

number of carbon atoms in longest chain
5_0__
There are five carbon atoms in the chain.

position of double bond
2_0__
Always number from the side that gives the double bond position the smallest number.

isomer (cis or trans)
o_trans_s
The carbon-carbon bonds adjacent to the double bond are on opposite sides of the double bond.

name
o_trans-2-pentene_s
A five-carbon alkene with the double bond after the second carbon and the C–C bonds on opposite sides of the double bond is trans-2-pentene.

number of carbon atoms in longest chain
6_0__
There are six carbon atoms in the chain.

position of double bond
3_0__
The double bond is after the third carbon in both directions.

isomer (cis or trans)
o_cis_s
The carbon-carbon bonds adjacent to the double bond are on the same side of the double bond.

name
o_cis-3-hexene_s
A six-carbon alkene with the double bond after the third carbon and the C–C bonds on the same side of the double bond is cis-3-hexene.

13.3-9. Stereocenters
A carbon atom with four different groups attached to it is called a stereocenter.
Exercise 13.8:
Is the carbon labeled in red a stereocenter?








13.3-10. Enantiomers
Enantiomers are isomers that contain stereocenters and are mirror images of one another.
molecule A in Figure 13.14 is a stereocenter because it contains four different groups: H, OH, CH3, and Cl. Molecule B in Figure 13.14 is its mirror image. Molecules A and B are different molecules! That they are different can be seen by rotating molecule B by 180° about the C–C bond (B1 → B2). The rotation makes the OH and CH3 groups of the two molecules (A and B2) superimposable. However, the H and the Cl groups are still reversed in the two molecules. Consequently, molecules A and B are not superimposable. Note that your right and left hands are also mirror images that are not superimposable. Molecules with a stereocenter cannot be superimposed on their mirror images. Pairs of molecules that are nonsuperimposable mirror images are called enantiomers. Enantiomers are stereoisomers because their spatial arrangements are different while their connectivities are identical. Molecules A and B are enantiomers.

Figure 13.14: Enantiomers
The two molecules each contain a stereocenter, and they are mirror images of one another. Consequently, they are enantiomers.
13.3-11. Enantiomer Exercise
Exercise 13.9:
The following molecule has a stereocenter.
Indicate whether each of the following molecules is the same molecule or its enantiomer.

13.3-12. Enantiomeric Compounds
Enantiomers have the same melting and boiling points, but they often have dramatically different biological activities because biological activities are often based on the structures of the reacting molecules. Thus, one compound may cause one effect, but its enantiomer may cause a very different effect or have no effect at all. All of the biologically active amino acids except one have stereocenters, and in each case, only one enantiomer is active. The following are a couple of examples.
Figure 13.15: Carvone
Carvone contains one stereocenter (the yellow carbon on a blue field). The enantiomer on the left is recognized by the olfactory receptors in the nose as the odor of caraway, while the one on the right is recognized as the odor of spearmint.

Figure 13.16: Thalidomide
Thalidomide contains one stereocenter (the yellow carbon on a blue field). One stereoisomer acts as a sedative and antinausea drug, while the other causes severe birth defects. A drug that contained both enantiomers was marketed in Europe between 1959 and 1962 and resulted in thousands of badly deformed babies. Today, the enantiomer that caused birth defects is being studied as a possible treatment for leprosy, AIDS, and cancer.
13.3-13. Counting Mirror Images
A large structural diversity can occur in molecules that contain several stereocenters. In general, there are 2n stereoisomers of a molecule with n stereocenters. Thus, there are 2 stereoisomers of a molecule with only one stereocenter, 22 = 4 stereoisomers of a molecule with two stereocenters, and 215 = 32,768 stereoisomers of a molecule with 15 stereocenters.
Exercise 13.10:
Consider the sucrose molecule, table sugar. The red highlight of one carbon atom is there for discussion purposes only. It is not special in any other way.

How many stereocenters does a molecule of sucrose contain?
9___
All of the carbon atoms that are members of either ring are stereocenters. For example, consider the carbon atom that is highlighted in red. While it is attached to two C atoms that appear to be identical, they are different due to differences arising from the ring structure. For example, the carbon that is attached to the bridging oxygen atom is the second atom you encounter when moving counterclockwise, but it is the fourth atom when moving clockwise. This difference is sufficient to make the C atom in red a stereocenter.

How many stereoisomers of sucrose are there?
512___
Sucrose contains nine stereocenters, so there are 29 or 512 stereoisomers, which would consist of 256 enantiomeric pairs.

13.4 Functional Groups
Introduction
Organic molecules are designed with specific sizes, shapes, and functional groups to perform specific functions (e.g., a better drug or a stronger plastic). Once a molecule is designed, organic chemists develop procedures to make it. These procedures, which can involve many steps, can be developed because so many organic reactions proceed by a few well understood mechanisms. Organic mechanisms are based on the principles of the octet rule, resonance, and Lewis acid-base theory. Reactions occur at specific groups within the molecule called functional groups. Functional groups have specific reactivities that can be utilized to produce desired reactions. In this section, we introduce some of the functional groups and the fundamental types of reactions they undergo.Objectives
-
•Identify some common functional groups.
13.4-1. Alcohols
Alcohols can behave as Brønsted acids because they contain a hydrogen atom attached to an electronegative atom, but they are also Lewis bases because the oxygen atom contains two lone pairs. Alkyl groups are usually electron donating, so alcohols in which R is an alkyl group function more as Lewis bases than acids.
Alcohols have the general formula R–OH, where R is a generic group of atoms and OH is the hydroxyl group.

Figure 13.17: Generic Alcohol
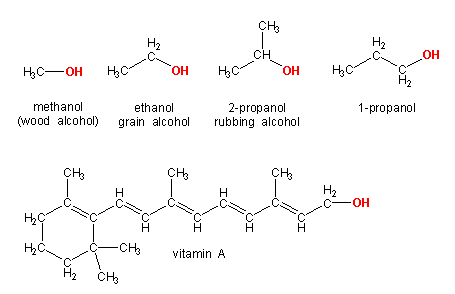
Figure 13.18: Some Common Alcohols
13.4-2. Carbonyls
The carbonyl group is C=O.

Figure 13.19: Resonance Forms of a Carbonyl Group
13.4-3. Carboxylic Acids
A carboxyl group is the combination of a carbonyl and a hydroxyl group.

Figure 13.20: Carboxylic Acid
A molecule with a carboxyl group is called a carboxylic acid (RCOOH).

Figure 13.21: Acidity of Acetic Acid
Replacing the O–H hydrogen of a carboxylic acid with an alkyl group results in an ester (RCOOR').

Figure 13.22

Figure 13.23
13.4-4. Amines and Amides
An amine is an ammonia molecule in which one or more of the hydrogens have been replaced with R groups. If the nitrogen is attached to a carbonyl, the group becomes an amide group.

Figure 13.24

Figure 13.25: Amides

Figure 13.26: Caffeine
13.4-5. Amino Acids
Amino acids contain an amine and a carboxylic acid.

Figure 13.27: Alanine, an Amino Acid
Note that the carbon atom in alanine that is connected to both the amine and carboxyl groups is a stereocenter. This is the case in almost all amino acids found in the human body. However, only one enantiomer is biologically active.
13.5 Introduction to Organic Reactions
Introduction
A good amount of organic chemistry can be understood with Lewis acid-base theory. Several mechanisms for different reactions are presented in this section, but all are Lewis acid-base reactions.Prerequisites
-
•5.6 Determining Lewis Structures (Draw Lewis structures.)
-
•5.8 Formal Charge and Oxidation State (Determine formal charge.)
-
•12.1-7 Curved Arrows in a Mechanism (Represent the mechanism of a Lewis acid-base reaction with curved arrows.)
-
•12.1 Lewis Acids and Bases (Determine Lewis acidic and basic sites.)
Objectives
-
•Identify the Lewis acidic and basic sites involved in an organic reaction.
-
•Describe an addition reaction.
-
•Describe a condensation reaction and use curved arrows to show the mechanism for the reaction of a carboxylic acid and an alcohol.
-
•Use curved arrows to show the attack of an amine on an ester and predict the product of the reaction.
13.5-1. Addition Reactions of Alkenes
Addition reactions are reactions in which two reactants combine to form a single product. We use the addition of a hydrogen halide across a C=C double bond as an example.The Addition of a Hydrogen Halide Across a Double Bond
- Viewing the Video
-
•View the video in this window by selecting the play button.
-
•Use the video controls to view the video in full screen.
-
•View the video in text format by scrolling down.

Figure 13.28: The Addition of a Hydrogen Halide Across a Double Bond
-
1The pi electrons of the double bond attack the hydrogen of the hydrogen halide to produce a C–H bond, a halide ion, and a carbocation.
-
2A lone pair on the halide ion attacks the strong Lewis acidic carbocation to form a C–X bond.
13.5-2. Esterification
In condensation reactions, two reactants combine to form two products (one of which is often a small molecule such as water). A carboxylic acid and an alcohol can undergo a condensation reaction called esterification to produce an ester and water.
Figure 13.29: Esterification
R and R' are usually two different alkyl groups.
Chapter 12. Indeed, only the identities of the Lewis acid and base have changed.
Esterification Video
- Viewing the Video
-
•View the video in this window by selecting the play button.
-
•Use the video controls to view the video in full screen.
-
•View the video in text format by scrolling down.
3D Animation of Esterification
- Viewing the Video
-
•View the video in this window by selecting the play button.
-
•Use the video controls to view the video in full screen.
-
•View the video in text format by scrolling down.
-
1In the first step, the lone pair on the oxygen of the alcohol (the Lewis base) attacks the carbonyl carbon of the acid (the Lewis acid) in a Lewis acid-base reaction. The lone pair becomes a C–O bond, and the pi electrons of the C=O double bond of the carbonyl become a lone pair on the oxygen.
-
2A water molecule from the solvent (shown in green) assists in a proton transfer. It first accepts a proton from the oxygen with positive formal charge and then donates a proton to the oxygen involved in the O–H bond.
-
3The proton transfer in the second step produces an OH2 group with a positive formal charge on the oxygen. Electron density moves out onto the oxygen with positive formal charge to produce a water molecule. The water molecule is lost and the C–O pi bond reforms, resulting in a molecule with no formal charge. Loss of small molecules such as water is common in condensation reactions.

Figure 13.30: Esterification Mechanism
13.5-3. Amide Formation
Amides can be formed in a Lewis acid-base reaction of an amine (base) and an ester (acid). The other product of this condensation reaction is an alcohol.
Figure 13.31: Amide Formation
Amide Formation Video
- Viewing the Video
-
•View the video in this window by selecting the play button.
-
•Use the video controls to view the video in full screen.
-
•View the video in text format by scrolling down.
3D Animation of Amide Formation
- Viewing the Video
-
•View the video in this window by selecting the play button.
-
•Use the video controls to view the video in full screen.
-
•View the video in text format by scrolling down.
-
1The lone pair on the nitrogen atom of the amine (the Lewis base) attacks the carbonyl carbon of the ester (the Lewis acid) in a Lewis acid-base reaction. The lone pair becomes a C–N bond, and the pi electrons of the C=O double bond of the carbonyl become a lone pair on the oxygen.
-
2A water molecule from the solvent assists in a proton transfer. It first accepts a proton from the nitrogen with positive formal charge and then donates a proton to the oxygen involved in the O–R' bond.
-
3The proton transfer in the second step produces a R'OH group with a positive formal charge on the oxygen. Electron density moves out onto the oxygen with positive formal charge to produce an alcohol molecule (R'OH), which is lost as the C–O pi bond reforms, resulting in a molecule with no formal charge. Loss of small molecules such as alcohols is common in condensation reactions.

Figure 13.32: Amide Formation Mechanism
13.6 Polymers
Introduction
Natural polymers, such as carbohydrates, proteins, and nucleic acids, are part of living tissue and are responsible for increasing the rates of reactions and giving structural characteristics like strength and flexibility. Others store our genetic code. When most of us think of polymers, however, we think of man-made polymers like PVC, teflon, polyethylene, or polyesters. In this section, we examine both natural and man-made polymers.Prerequisites
-
•7.3-10 Hydrogen Bonding (Describe the hydrogen bond and state the requirement for it.)
Objectives
-
•Define monomer, dimer, trimer, and polymer.
-
•Identify the monomer upon which a polymer is based.
-
•Recognize some common polymers.
-
•State the requirement for the reactants used to produce a condensation polymer.
-
•Distinguish between a polyamide and a polypeptide.
-
•Explain why proteins frequently form α-helices.
-
•Describe a base pair, explain why they are important, and identify the hydrogen bonding sites in a base pair.
-
•Describe the double helix structure of DNA and explain its origin.
Addition
13.6-1. Polymers
Polymers are large molecules consisting of many single unit building blocks called monomers.

Figure 13.33: Several Mers
13.6-2. Addition Polymers
Addition polymers are formed in addition reactions.

Figure 13.34: Alkene Polymerization
Polymer | Structure | Uses |
---|---|---|
polyethylene | ![]() |
plastic bottles and bags |
propylene | ![]() |
carpets |
isobutylene | ![]() |
tires |
poly(vinyl chloride) (PVC) |
![]() |
plumbing and hoses |
polystyrene | ![]() |
insulation |
tetrafluoroethylene (teflon) |
![]() |
cooking utensils and nonstick cooking surfaces |
Table 13.6: Structures and Uses of Some Common Polymers
13.6-3. Isobutylene
The reaction of isobutylene with hydrogen halides was used as an example of an addition reaction in the previous section. We now examine the mechanism of the reaction.Isobutylene Polymerization Mechanism
- Viewing the Video
-
•View the video in this window by selecting the play button.
-
•Use the video controls to view the video in full screen.
-
•View the video in text format by scrolling down.
3D animation
- Viewing the Video
-
•View the video in this window by selecting the play button.
-
•Use the video controls to view the video in full screen.
-
•View the video in text format by scrolling down.

Figure 13.35: Polymerization of Isobutene into Isobutylene

Figure 13.36: Isoprene
Condensation Polymers
13.6-4. Condensation Polymers
Condensation polymers are produced in condensation reactions.
x diacid + x diol → polyester

Figure 13.37a: Polyester Poly(ethylene terephthalate) or PET: Step 1
The reaction of a diacid and a diol produces an ester that contains a hydroxyl group at one end and a carboxyl group at the other.

Figure 13.37b: Polyester Poly(ethylene terephthalate) or PET: Step 2
The ester formed in step 1 can react with another diacid molecule and another diol molecule to produce a compound with three ester linkages (the original one and two new ones, which are circled). The product still contains a hydroxyl group at one end and a carboxyl group at the other end, which can react with more diacid and diol molecules.

Figure 13.37c: Polyester Poly(ethylene terephthalate) or PET: Product
Continued reaction increases the number of ester linkages, but maintains one hydroxyl group and one carboxyl group that can react. The monomer unit of the PET polymer is shown.
13.6-5. Nylon 66
Nylons are polyamides formed from the reaction of a diester and a diamine.
x diamide + x diester → polyamide (nylon)
-
1Lone pairs on N atoms of diamines (red and blue in Figure 13.38a) attack carbonyl carbon of diester to form C–N bonds.
-
2Proton transfers (shown in circles in Figure 13.38a) from diamine nitrogens to oxygens of diesters produce CH3OH groups that leave as methanol molecules.

Figure 13.38a: Nylon

Figure 13.38b: Nylon 66
13.6-6. Polypeptides
Polypeptides are polyamides formed by the reaction of amino acids.

Figure 13.39a: Polypeptides: Dipeptide
Two amino acids (glycine and alanine or GLY-ALA) combine to form a dipeptide. The resulting dipeptide still has a reactive site at each end (an amine and a carboxylic acid).

Figure 13.39b: Polypeptides: Tripeptide
Three amino acids (glycine, alanine, and serine or GLY-ALA-SER) combine to form a tripeptide. Alternatively, a serine molecule reacts with the dipeptide formed in the first step.
13.6-7. H-Bonding in Proteins
Proteins are large polypeptides.

Figure 13.40: H-Bonds Responsible for the α-helix Structure
13.6-8. Protein Structure
An α-helix is shown in Figure 13.41. Color code: red = oxygen, blue = nitrogen, gray = carbon, white = hydrogen, and yellow = sulfur. Note only those hydrogen atoms involved in hydrogen bonding are shown.- (a) Space-filling model
- (b) Ball-and-stick model with green ribbon to show polypeptide backbone
- (c) Ball-and-stick model in which the side chains have been removed, and hydrogen bonds are shown as dashed lines

Figure 13.41: Representations of an α-Helix
DNA
13.6-9. Nucleotides
A nucleotide consists of a phosphate, a sugar, and an N-containing base.
Figure 13.42
The sugar in this nucleotide is called deoxyribose.

Figure 13.43: Three Residues of a DNA Molecule
13.6-10. Bases
Only four bases, which exist in two base pairs, are found on the nucleotides that form DNA.

Figure 13.44
13.6-11. Determining Base-Pairs Exercise
Exercise 13.11:
Adenine and guanine do not interact favorably, so they are not base pairs. Determine the base pair of each (T or C) based on how well each interacts with one of the other base pairs.
base pair of guanine (T or C)
o_C_s
Cytosine aligns with guanine to provide three hydrogen bonding sites. H–H interactions keep guanine from interacting favorably with thymine. Thus, cytosine and guanine (C–G) form a base pair.


base pair of adenine (T or C)
o_T_s
Adanine aligns with thymine to provide two hydrogen bonding sites. H–H interactions keep adanine from interacting favorably with cytosine. Thus, thymine and adanine (T–A) form a base pair.


13.6-12. Base Pairs
Hydrogen bonding between base pairs results in the double helix structure of DNA.
-
1adenine (A) - thymine (T)
-
2guanine (G) - cytosine (C)
strand 1 with the cytosine on strand 2 hold the two strands of DNA in this portion in a double helix.

Figure 13.45: Base Pairs in DNA
13.6-13. Double Helix and Function of DNA
The structural unit of DNA is called the double helix. The double helix is a consequence of hydrogen bonding between base pairs on different strands. It is the sequence of the base pairs in the double helix that forms the genetic code. Thus, your genetic code requires only four different chemical letters: A, T, C, and G. However, the human cell contains about three billion base pairs.
Figure 13.49: Double Helix of DNA